Kilometre-Scale Modelling of the Earth System: A New Paradigm for Climate Prediction
Global climate modelling has made great strides over the last 50 years. Simple energy balance models evolved into coarse resolution General Circulation Models, then into the coupled Earth System Models (ESMs) we have today.
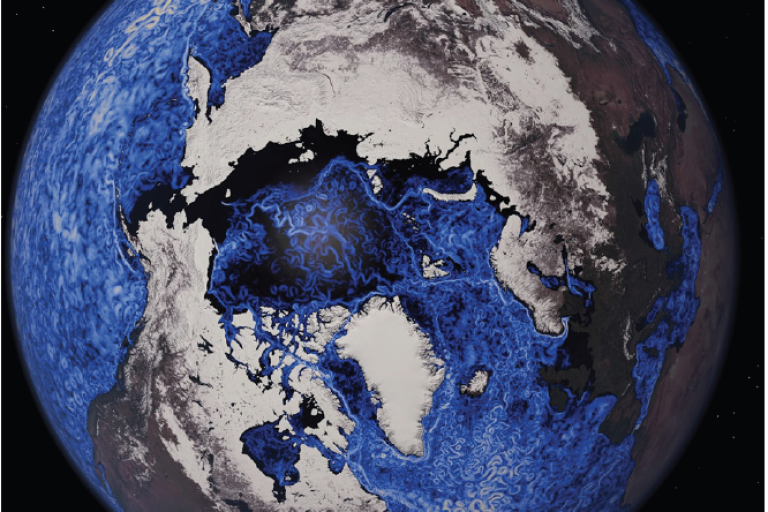
By Andrew Gettelman1, Bayler Fox-Kemper2, Gregory Flato3, Daniel Klocke4, Detlef Stamer5, Bjorn Stevens4 and Pier Luigi Vidale6
Global climate modelling has made great strides over the last 50 years. Simple energy balance models evolved into coarse resolution General Circulation Models, then into the coupled Earth System Models (ESMs) we have today. These ESMs include many spheres – atmosphere, ocean, cryosphere – as well as the chemistry and biology of the carbon cycle and the hydrology of the water cycle, and can project broad outlines of the future climate. However, the costliest impacts of climate change will be felt via local and regional extremes, such as extreme weather, multi-year drought and inundation from sea-level rise combined with storm surge, which these models cannot project. But adaptation to climate change requires local information on these threatening risks. Driven by these imperatives, a new class of regional and global ESMs are now emerging. These “km-scale” models have horizontal scales of less than 10 kilometres (< 10 km). Computing advances promise the possibility of global km-scale simulations a century long within the next five years – but several challenges lie ahead.
Conceptually, climate and Numerical Weather Prediction (NWP) models are similar: they often share a dynamical core and key parameterizations such as radiation and turbulence. The complexity and fidelity of the representation of physical processes in climate models is also comparable to, or higher than that of, NWP models. Many global centres – Met Office (United Kingdom), European Centre for Medium Range Weather Forecasts (ECMWF), Deutscher Wetterdienst (DWD) – have “unified” models that can simulate either weather or climate using data assimilation in the weather prediction context. However, the longer timescales of interest in climate modelling demand an active ocean circulation, an evolving land biosphere, hydrosphere, cryosphere and balanced global energy and carbon budgets. These components are necessary to simulate how features like soil moisture, permafrost, sea-ice and snow cover will change.
Ensembles of climate models, such as described in the article Climate projections in next phase of the Coupled Model Intercomparison Project (CMIP) in this issue, have been repeatedly used to create large-scale projections of global climate. As a result, the broad outlines of climate futures are known and generally accepted. However, the regional and local impacts of that future climate continue to have large uncertainties, driven by uncertainty in models (structural uncertainties and unrepresented processes) and by natural variability, which plays a larger role at the regional scale. Km-scale modelling promises fewer unrepresented processes and more accurate regional modelling.
Currently, climate risks and impacts are beginning to be felt around the world, with the relative weights of hazard (weather event), exposure (scale of human infrastructure) and vulnerability (resilience or lack thereof) changing rapidly as human activities evolve. The impacts are already being observed through local extreme events. These include temperature and precipitation extremes from atmospheric blocking, such as the 2021 Pacific Northwest heat wave in North America, an increase in the rapid intensification of tropical cyclones, like Hurricane Otis in 2023, and flooding from pluvial, riverine and cryospheric origin, such as the 2023 glacial lake outburst flood in India. The frequency and intensity of many hazards and exposure are expected to grow over time, but the goal is to reduce impacts by increasing resiliency through adaptation. Optimizing adaptation to meet future extremes requires a new approach.
In the past, the large computational power required for climate modelling permitted only coarse global resolutions for climate simulations. Because of these limitations, models were only able to resolve the storms of the extratropics, and many features like tropical weather systems had to be parameterized. To fill the need for local information, mesoscale weather models were adapted into “regional climate models” and sometimes even coupled with local ocean or sea-ice dynamics to capture maritime and polar climates. These regional climate models addressed the gap for local scale information for extreme weather and impacts under climate change and are still today a common approach for adaptation studies.
The provision of boundary conditions in regional models comes from global climate change simulated with large-scale climate models or with historical weather “perturbed” by average climate change from large-scale models. The regional approach makes fundamental assumptions about the climate system and, therefore, has inherent limitations. For instance, regional climate models inherently create a scale “break” when downscaling large-scale forcing from global models to define regional boundary conditions. Moreover, these regional models do not have “upscale” propagation of small phenomena that create upscale climate impacts. For example, oceanic mesoscale eddies tend to drive atmospheric mesoscale storms in the extratropics, while at larger scales the atmosphere tends to drive ocean variability. These effects are apparent only in coupled systems and their large-scale consequences, such as the orientation of the storm tracks and related air-sea fluxes, can only be captured in large-domain models with mesoscale or better resolution. Regional models cannot simulate these upscale effects.
Furthermore, regional modelling efforts are still usually of coarse resolution (10–25 km horizontal resolutions), which is insufficient for simulating many phenomena that drive regional extremes. Finally, regional climate models are often custom built for a particular region, with limited applicability or testing beyond that region.
A new class of models
The new class of regional and global Earth system km-scale models credibly simulate the dynamics of extreme weather and their driving factors over large domains. An example of a test simulation of a global model at 1.25 km horizontal resolution is offered in Figure 1: at left is the first full global photograph of the Earth taken by Apollo 17 in 1972 (the “Blue Marble” photograph), next to it is the test simulation by the global model. But these models are computationally expensive. Currently, we can run 10 km global models for centuries, 3 km global atmosphere or ocean models alone for a few years, and 1 km global atmosphere models for only a few days, but these capabilities are changing rapidly.
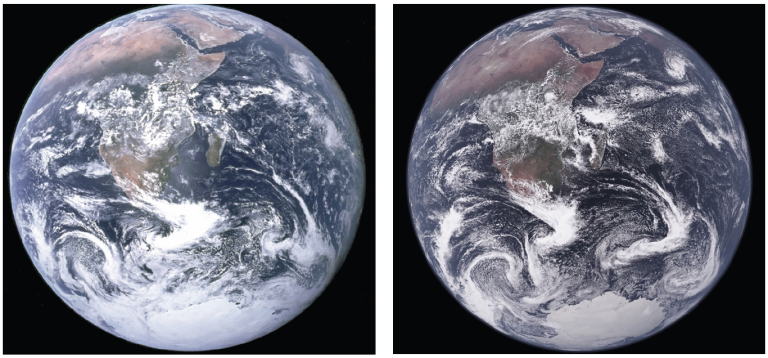
The availability of “exascale” – capable of 1018 floating point operations per second – computing resources, a significant portion of which is earmarked for weather and climate applications, offers unprecedented opportunities. Three-km global simulations at the centennial scale will be increasingly feasible for many within the next five years. Significant advances in software and in computational efficiency have been as important, or more, than advances in hardware provision.
At km-scale, orography and bathymetry can capture important features controlling small-scale climate. Increasing resolution removes the need to parameterize many processes, for which the community has struggled to find an adequate statistical treatment. Now it is possible to resolve the weather systems that influence the tropics, and small-scale summer storms in the extratropics, with the same approaches used to resolve the major storms of the extratropics. In the ocean, mesoscale internal instabilities are permitted at 10–25 km resolution, but at scales near 3 km the oceanic submesoscale fronts and instabilities are also permitted and can interact with important narrow regions of the cryosphere such as the Marginal Ice Zone and the ice shelves that buttress the Antarctic Ice Sheet.
Of course, not everything will be resolved, as oceanic and atmospheric small-scale turbulent processes or evapotranspiration in leaves will still require more approximate and uncertain treatments. At km-scale resolution important processes are explicitly represented, like vertical atmosphere motions that drive cloud physics, ocean submesoscale fronts and eddies, sea-ice leads, and ice shelves. While working at km-scales will bring a new set of challenges for representing the scales still unresolved, it allows for a closer link to observational communities working at similar scales.
Recent comparative studies among km-scale ocean models show that the large-scale features that affect the storm tracks and air-sea coupling (e.g., Gulf Stream separation) are much more consistent than in coarser models. Internal variability is also substantially larger in these eddy-rich models. Figure 2 illustrates the speed of the surface current in the ocean and the snow cover from the 1 km simulation in Figure 1. Recent work has shown that extratropical oceanic mesoscale variability, now resolved in these models, drives maritime atmospheric variability unlike at larger scales, where the atmosphere drives the ocean. Near the grid-scale, however, these models exhibit great diversity in turbulence intensity. This is because smaller submesoscale features, important for air-sea coupling and biogeochemistry, are still not resolved at km-scales and remain highly sensitive to parameterizations.
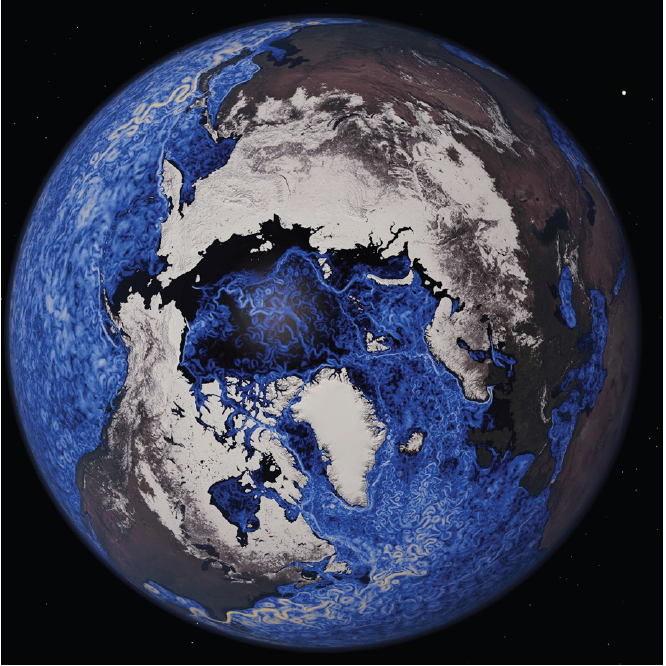
Comprehensive models are needed to integrate climate impacts at the regional scale, which can have global implications. For example, critical interactions of the warming oceans and the Antarctic ice shelves – which create uncertainty about the potential for sudden sea-level rise and meridional overturning shutdown – will be directly addressed at km-scale resolution. However, that will require representations of ice sheets and glaciers. Other impacts, such as flooding from precipitation extremes, will require representation of atmosphere-surface interactions, soil moisture and groundwater movement, and frozen ground in cold climates. Sea ice, on the km-scale and below, may have to be represented differently than at larger scales. The inclusion of such phenomena may require improvement or alterations to existing component models for km-scale applications, the representation of currently missing processes, or even new component models (e.g. rivers, waves), but offer the opportunity to work much more closely with observational communities working on similar scales.
There are many critical areas where development is required in these models, necessitating interdisciplinary teams. In the ocean, km-scale models directly resolve mesoscale eddies that are important for driving both oceanic and atmospheric extremes on small scales. Better resolution of the complex bathymetry of narrow straits important for ocean circulation would resolve upwelling regions critical for coastal fisheries and for credibly simulating the local consequences of the El Niño/La Niña Southern Oscillation (ENSO). Higher resolution resolves small islands, enabling direct assessment of sea-level and coral ecosystem impacts. The land surface at high resolution can now also resolve the evolution of water from the atmosphere into soil and back to the surface, enabling complex hydrology. Lateral flow of water under the surface must be represented. Sea-ice models at km-scale can represent large leads and polynyas and can explicitly resolve marginal ice zone processes.
Regional models are also pushing towards the km scales. This includes active efforts in many regions, including Africa and South America facilitated by collaborations with centres in the United Kingdom (UK) and United States of America (US) respectively. Hybrid approaches, wherein a global model has a regionally refined or nested high-resolution region to maintain fidelity but reduce computational load, may also accelerate development and use of km-scale models. These can potentially gain the benefits of global km-scale for some applications, but at reduced cost as a bridge to global km-scale.
Opportunities
Km-scale ESMs present a new opportunity to make substantial rather than incremental progress in understanding climate extremes, circulation changes, and their local impacts on society. These models do not solve every problem, but they do remedy many that have limited progress in the past, such as resolving the mesoscale organization of storms, critical for precipitation extremes. Significantly, the problems that these models do not solve are now posed in ways that might allow us to solve them. For example, km-scale models, like NWP models, can be more easily compared to a wealth of observations of weather phenomena, rather than just approaching such evaluation statistically. New sources of data, such as active radar and lidar in space, are being taken at the scale of these models.
Regional models enable easier access to information, on a scale comparable to observations, but they truncate scale interactions and are often not comprehensive in their Earth system processes. For example, regional models and weather models often do not include proper radiation balance, carbon cycling, coupling to ocean and cryosphere, etc. Also, km-scale regional refinement, embedded in global models, can provide many of the benefits at much more accessible cost, and limited regional modelling backed by global simulations can still fill in gaps.
The importance of representing extremes, circulation changes and societal needs for information on local climate impacts also require new paradigms for using and analysing these models. Developments are taking advantage of new paradigms for open science, open data, data science and machine learning to accelerate models and progress. Working together and beyond the physical sciences – thereby involving more nations and peoples whose modelling capacity is underdeveloped – offers big opportunities. Some climate modelling centres have already taken on such partnerships and are actively working with a broad array of stakeholders.
Rising to the challenges
Km-scale ESMs face many challenges. One issue is procuring suitable observational data to serve as initial and boundary conditions (particularly in the ocean, cryosphere, and continental subsurface). Then, though such high-resolution models have the potential to greatly reduce model error and improve process representation, the currently short simulations make verification of slow components harder – fast components like the atmosphere can be verified, similarly to NWP models, on past weather. Data assimilation methods offer exciting possibilities for initializing slow components (ocean) in km-scale models. In addition to the formidable computing challenge for integrating the models, there are challenges in working with and analysing the output from km-scale models. These challenges require adoption of several new paradigms all at once.
As with every new type of model, km-scale models expand the frontiers of human inquiry. Traditional computational approaches have met their limits, particularly around energy efficiency. The use of hardware accelerators (like graphical processing units) and emulators with Artificial Intelligence/ Machine Learning (AI/ML) methods is starting to significantly speed up (i.e., reduce the cost of) integration and analysis. AI/ML will enable new approaches around emulation, data compression, data assimilation and process acceleration. These new approaches will be required for these models to be useful. Traditional approaches of running a climate model once, saving everything, then moving and is too much data for long-term storage or to transfer to many different other systems. New workflows and new analysis methods are now being developed in projects like DestineE (Europe) and Pangeo (US) to allow users around the world to interact with what would otherwise be overwhelmingly large amounts of data. Projects like NextGEMS (EU), together the establishment of community infrastructure access through facilities such as JASMIN (UK) and by the DKRZ (Germany), are also exploring ways in which to create public space within the digital sphere (a public cloud) that can share the benefits of these new opportunities.
Km-scale models coupled with human system models will enable us to provide a much richer spectrum of information. Ways to regularly deliver the best information to the people that need it will have to be identified. Climate change is here, and we know a lot about it, but it is all encoded in our models. That information needs to be decoded and delivered to those communities that need it. For example, modellers can learn from data systems developed to deliver satellite observations at similar km-scale, including data provided in the cloud (Google Earth Engine being an example from the private sector). There is a great opportunity as we move towards km-scale models to develop common approaches and to standardize the provision of information as “operational” climate services.
What is being done to improve?
Currently, km-scale models are developing rapidly. There are new paradigms for computation to separate scientific and technical concerns, which would make developing and advancing models less complex and more universal. New paradigms for data creation, archiving and analysis are evolving rapidly to a more just-in-time model of creating only the data that is needed, and accessing it where it is computed, without copying.
Km-scale models provide an active way to create counterfactual futures, and with common methods and analysis frameworks. In addition, as we build these models transition to an operational mode of data delivery, with rigorous aims for products, co-developed with stakeholders, and with testing and validation is imperative. These efforts need to be supported by strong research.
WCRP can promote, develop and provide that research backbone. For example, its Digital Earths “Lighthouse Activity” is helping advance km-scale model development by building communities around model development, analysis and research, including all components of the Earth system – atmosphere, land, ocean, cryosphere – as well as the human system. The goal is to help coordinate research activities that will support operational use of high-resolution global and regional climate models. The new Earth System Modelling and Observations (ESMO) core project will bring together observers and modellers to share experiences on archiving, analysing (including by AI) and sharing peta- and exascale datasets.
Interaction with stakeholders for climate information is advancing with individual case studies for application of km-scale models. But better structures are needed for the delivery of climate services that meet societal needs for climate information. There are several fine examples around the world of delivering operational climate data in a regular and validated way – like the standards for the delivery of “actionable” impact-based early weather warnings. Continued development is needed for km-scale information. The Earth Visualization Engines concept, for example, proposes a new framework for delivering climate information with improved cloud access through public infrastructure, and improvements in the interoperability of software and data.
Km-scale models can meet key societal needs for simulations of extremes that consider all scale interactions and that can be verified against advanced observations. Km-scale models can also unleash the power of co-development of climate services with stakeholders at regional and local scales, make full use of exascale computing opportunities and pioneer access to an open public space for climate data.
Footnote
1 Pacific Northwest National Laboratory, USA
2 Department of Earth, Environmental & Planetary Sciences (DEEPS), Brown University
3 Environment and Climate Change Canada (ECCC)
4 Max Planck Institute for Meteorology
5 Centre for Earth System Research and Sustainability (CEN), Hamburg University
6 National Centre for Atmospheric Science (NCAS), Department of Meteorology, University of Reading